Supernova
1987a
On the night of February 23, 1987 astronomers saw
something they hadn't seen for 400 years...
a supernova explosion close enough to be seen with the naked
eye. A massive "blue giant"
star, 50 times as large as our sun, had exploded in the Large Magellenic
Cloud (a small suburb
of our galaxy). The explosion actually occurred 170,000 years before...
it took that long for the light to get here.
When a large star has burned up all of the nuclear
fuel in it's center it becomes,
in a few seconds, an almost empty shell and suddenly collapses.
The rebounding matter and energy becomes a very dense, and very
bright, source of light.
Suddenly the object becomes hundreds of time brighter than its progenitor
star.
The "before"
and "after" pictures for SN1987a are shown below.
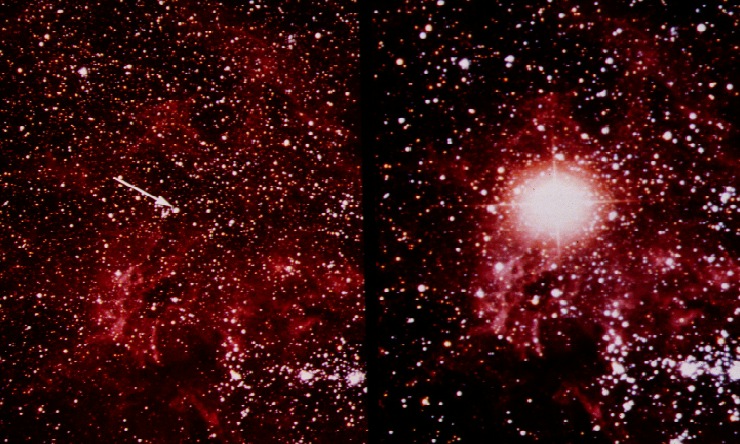
The arrow on the left shows the progenitor star,
Sanduleak -69 202.
The picture at right shows it shortly after the explosion
during which it increased its luminosity by a factor of 100 million.
(Most of the other bright objects in the photo are ordinary stars
like our sun.)
During the first few seconds of such an explosion
the temperature near the center is so
hot (10^10 deg) that huge numbers of neutrinos, electrons, and photons
are created.
The weakly interacting neutrinos are the only ones that can escape
easily so they carry away
most of the explosion energy.
The number of neutrinos emitted is extremely large...
about 10^57 escape in a few seconds.
After 170,000 years this pulse of neutrinos is spread out over the
surface of a sphere
170,000 light-years in radius.... big enough to encompass our whole
galaxy.
Spreading out the 10^57 neutrinos over the surface
of this huge sphere gives 10^13 neutrinos
per square meter. All of the neutrinos are contained in a thin shell
on the surface. The shell is only
a few light-seconds thick (about the distance from here to the moon).
Of the 10^16 neutrinos that went through the IMB
tank only 8 interacted with enough energy
to be detected.... all near the lower limit of our energy threshold.
The normal rate of events from atmospheric neutrinos at these low
energies was only about one
per week, so seeing 8 in a few seconds meant something truly unique
had happened.
Pictures of one of the events
in the IMB detector are shown below.
To see pictures of all eight
of the events click here
|